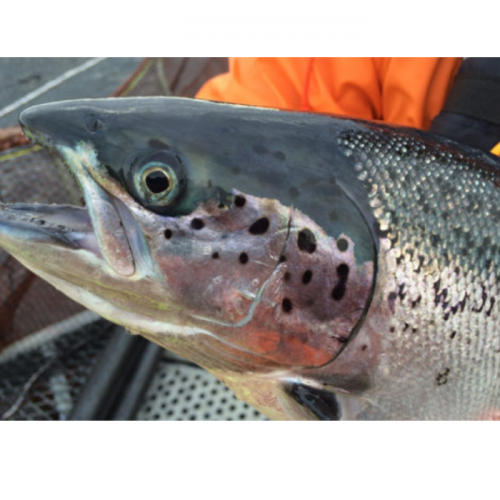
Genome Editing: Potential to Improve Aquaculture Breeding, Production
| Tue, 24 Sep 2019 - 14:36
Need for sustainable aquaculture production, current status of genome editing
The CRISPR/Cas9 genome editing technology has already been successfully applied to several aquacultured species, including Atlantic salmon and Pacific oysters. Photos by Darryl Jory.
The role of aquaculture in food security
Food security is a major and increasing global challenge, associated with a rapidly growing demand for high-quality animal protein. Competition for land use will present a serious limitation to the scope for increases in terrestrial crop and animal production. Therefore, it is likely that aquaculture will have a growing role in meeting this rising food and nutrition demand. Fish production via aquaculture is now approximately equal to capture fishery production for the first time in history, will be the dominant source of seafood within a few decades, and is the fastest growing food production sector, predicted to grow by 31 percent over the next 10 years.
Fortunately, development potential is huge, with only ~1 percent of suitable marine sites currently being used for aquaculture. Furthermore, aquaculture production is considered efficient in terms of feed conversion and protein retention compared with most terrestrial livestock, and seafood is the major source of long-chain polyunsaturated fatty acids, which are considered essential for human health. However, relative to many crop and livestock production systems, most aquaculture is at a formative stage and is typically a high-risk activity. Sustainability can be hindered by an initial lack of control of the reproduction cycles of species, and periodic collapses due to infectious diseases. Upscaling and improving the reliability of production will require disruptive innovation in engineering, health, nutrition and genetic improvement technologies, the latter being the focus of this review.
Genetic improvement for sustainable aquaculture production
Domestication and genetic improvement of terrestrial livestock has occurred for several millennia, with organized breeding programs for most species in place for more than 50 years. The results have been striking; for example, selective breeding has led to a threefold increase in the efficiency of milk production in cows, with similar gains for other target traits. By contrast, relatively little aquaculture production is underpinned by modern selective breeding programs.
Most farmed aquatic species are either still sourced from the wild or in the early stages of domestication, suggesting that there is substantial standing genetic variation for traits of economic importance. The reproductive biology of aquatic species can be amenable to the application of genetics and breeding technologies, enabling high selection intensity and, therefore, genetic gain. In part, this is due to the near-universal high fecundity of aquatic species, and the resulting large nuclear families, which can facilitate extensive collection of phenotypic records in close relatives (including full siblings) of selection candidates in breeding programs.
The reproductive output from genetically improved broodstock together with ease of transport of eggs and juveniles, also means widespread dissemination of improved stocks can have a rapid impact on production. Furthermore, with the development of high-density SNP arrays [a SNP array is a microarray platform that provides the genotype of an individual for many thousands of SNPs (single base-pair differences in DNA sequence at a specific region of the genome) dispersed throughout the genome] and routine genotyping by sequencing genomic selection – the use of genome-wide SNPs to predict breeding values of selection candidates in a selective breeding program and to help inform which individuals to select for breeding – has become the state-of-the-art in several globally important aquaculture sectors, offering higher selection accuracies than selection based on phenotypic and pedigree records alone.
However, genetic progress in selective breeding is limited by the heritability of the target traits, the generation interval of the species and the need to target multiple traits in the breeding goal. In addition, advanced breeding programs are typically closed systems, and are limited to the standing genetic variation in the broodstock (typically sourced from a limited sample of wild populations), and new variation that arises from de novo mutations. Genome-editing technologies – such as CRISPR/Cas9 (CRISPR stands for clustered regularly interspaced short palindromic repeats and Cas9 stands for CRISPR-associated protein 9 (CRISPR sequences together with the Cas9 enzyme can be used to make targeted changes to a genome) – offer new solutions and opportunities in each of these areas.
Advances in genome-editing technologies: CRISPR/Cas9 as the game-changer
In contrast to transgenesis, which involves the transfer of a gene from one organism to another, genome editing allows specific, targeted and often minor changes to the genome of the species of interest. Initial progress using other technologies has been largely superseded by the advent of the repurposed CRISPR/Cas9 system. The CRISPR/Cas9 system was discovered in bacteria, and was engineered to enable easy, cheap and efficient targeted editing of the genome. The system enables imperfect or targeted repair to create alterations to the sequence of the genomic DNA.
There are two primary repair mechanisms, each of which can be used to introduce different types of edit to the target genome. First, the two adjacent strands of DNA can be repaired through a technique called nonhomologous end-joining pathway (NHEJ), which is error-prone and induces insertion or deletions of a few nucleotides. Second, if a repair template is present, another technology called homology-directed repair (HDR) can be used to insert desired mutations (from a single nucleotide swap to a whole chromosomal region insertion).
Over the past few years, technical developments have made genome editing more efficient and raised new possibilities for biological discovery. There have also been numerous innovations that have enabled improved precision of editing, with lower off-target rates, and broadening of the range of target sites accessible via alternative Cas9 proteins. Novel extensions of the CRISPR/Cas9 editing system now allow researchers to better achieve gene activation or inhibition, and some techniques have the potential to target almost two-thirds of human SNPs.
Current status of genome editing in aquaculture species
Genome editing using CRISPR/Cas9 was recently successfully applied in vivo and/or in cell lines of several major aquaculture species like Atlantic salmon and rainbow trout); carps (Rohu, grass, and common carp); channel and southern catfish), as well as Pacific oyster, Nile tilapia and gilthead sea bream (Table 1). One major group of aquatic species where successful CRISPR/Cas9 editing has not yet been reported is shrimp (Penaeus sp.), which may be partly due to practical limitations, as discussed briefly below.
Most studies have a proof-of-principle focus, have typically followed CRISPR/Cas9 protocols developed in model organisms – such as zebrafish –and have often targeted genes with a clearly observable phenotype to test editing success (e.g., pigmentation). The standard methodology to induce in vivo mutations in aquaculture species is injection of the CRISPR/Cas9 complex into newly fertilized eggs as close as possible to the one-cell stage of development. Typically, mRNA encoding the Cas9 protein is injected together with the guide (g)RNA, leading to a high efficiency of editing that has been demonstrated in various species to date ; using the Cas9 protein in place of mRNA is also effective.
Target production traits for genome-editing studies in aquaculture species to date have included sterility, growth, and disease resistance. Creating sterile animals for aquaculture is desirable to prevent introgression with wild stock and to avoid the negative production consequences of early maturation. In this context, CRISPR/Cas9 has been used to induce sterility in Atlantic salmon and catfish. For growth-associated traits, several groups have edited the myostatin gene (famous for its role in double-muscled cattle, such as the Belgian Blue), resulting in larger fish. To date, this has been performed in channel catfish and common carp. Immunity and disease resistance have already been investigated using genome editing in rohu carp and grass carp, respectively, and it is expected that this area of research will flourish as a route to improving and understanding disease resistance as a key target trait for aquaculture.
Genome editing can also be applied to develop models for studying fundamental immunology, such as the targeted disruption of the TLR22 gene in carp. Such models can improve our fundamental understanding of host response to infection in fish and may lead to more effective treatment protocols. Along similar lines, it is plausible to use genome-editing technology to generate improved cell lines for fish species, for example by enabling more efficient production of viruses for future vaccine development by knocking out key components of the interferon pathway.
Genome editing to induce sterility and prevent wild introgression in Atlantic salmon: A case study
Most Atlantic salmon are farmed in open sea-cages, a production method that faces sustainability challenges, such as disease transmission from wild to farmed fish and vice versa, as well as escaped farmed fish impacting wild populations. A possible solution to these problems is the creation and use of sterile salmon in production. Currently, the only method available to sterilize commercial-scale numbers of salmon is triploidization (production of animals with three copies of every chromosome instead of the normal two). However, triploid (infertile) salmon are generally more sensitive to suboptimal rearing environments, which can make them prone to deformities and less tolerant to rising seawater temperatures.
There are two significant additional benefits of using sterile fish. First, early maturation is prevented, which avoids the associated negative phenotypes, such as reduced growth, lower flesh quality and higher susceptibility to disease. Second, sterility in production fish may safeguard Intellectual Property for the breeding companies. The gene encoding dead end (dnd) has been targeted to induce sterility in salmon, preventing the formation of germ cells. This was done using targeted mutagenesis (a process that changes the genetic information of an organism, resulting in a mutation) against dnd with CRISPR/Cas9, thereby creating a gene-edited sterile fish. Germ cell-free salmon will be 100 percent sterile and do not enter maturity.
Practical application of such sterile fish in breeding programs will require developments in genome editing, including knock-in, which could lead to the production of an inducible on-off system for sterility. Such mechanisms have been developed for the model fish species medaka and zebrafish. Use of this sterility technology may foster the future development of genome editing for other traits, such as disease resistance, with negligible risk of escapees interbreeding and passing edited alleles (variant forms of a given gene) on to wild stocks.
Some practical reasons why genome editing has such potential for research and applications in aquaculture species are the ease of access to many thousands of externally fertilized embryos, and the large size of those embryos facilitating microinjection by hand. The ability to use large nuclear families enables a degree of control of background genetic effects, with ample sample sizes achievable for downstream comparisons of successfully edited individuals with their unedited full-sibling counterparts. The ability to perform extensive “phenotyping” is often also feasible, for example using well-developed disease challenge models to assess resistance to many viral and bacterial pathogens during early-life stages. Finally, should favorable alleles for a target trait (e.g., disease resistance) be created or discovered, then there is potential for widespread dissemination of the improved germplasm for rapid impact via the aforementioned selective breeding programs.
In parallel, high-quality, well-annotated reference genomes are available for most of the key species. A high-quality species-specific reference genome is essential for the effective design of target guide RNAs (gRNA; one of two components of engineered CRISPR systems) with high specificity and minimum change of off-target editing, in particular given the relatively recent whole-genome duplication events that are features of several finfish lineages, including salmonids.
Integration of genome-editing technologies into aquaculture breeding and dissemination programs
If the public and regulatory landscape permits, genome-editing technologies are likely to be used in commercial aquaculture breeding in the coming years. However, for widespread adoption, maximal benefit, and minimal risk, it is necessary that these technologies are seamlessly integrated with well-managed selective-breeding programs. Achieving this will help ensure careful management of genetic diversity and avoidance of potential inbreeding depression.
In practice, the mass delivery of CRISPR/Cas9 to edit production or multiplier animals is unlikely to be feasible, and editing entire broodstock populations to carry the desirable alleles in the germplasm (living genetic resources like seeds or tissues that are maintained for the purpose of animal and plant breeding, preservation, and other research uses) is more practical. As such, inducible editing targets may be required for impacts on traits related to sterility and maturation.
In addition, technology developments are required to effectively integrate multiple edits simultaneously into broodstock animals to target multiple traits, or multiple causative alleles for the same trait. Thorough testing of edited animals is required to assess and exclude possibilities of unintended and potential detrimental pleiotropic effects of edits before any application in production.
However, once these issues have been addressed, widespread and rapid positive impacts could be achieved, because the high fecundity of most aquaculture species may enable dissemination to production systems without the need for pyramid breeding schemes typical of terrestrial livestock species.
Applications of genome editing for aquaculture research and production
Infectious diseases are one of the primary threats to sustainable aquaculture, with an estimated 40 percent of the total potential production lost per annum. Due to the formative stage of domestication of many aquaculture species, new selection and disease pressures in the farm environment may increase the possibility that standing genetic variation in farmed populations includes loci of major effect, which may represent potential low-hanging fruit for genome editing to increase the frequency of the favorable allele.
A well-known example of a major quantitative trait locus [QTL; a locus (section of DNA) which correlates with variation of a quantitative trait in the phenotype of a population of organisms]; often an early step in identifying and sequencing the actual genes that cause the trait variation.) affecting disease resistance is the case of infectious pancreatic necrosis virus (IPNV) in Atlantic salmon, in which a major QTL explains the majority of the genetic variation. Marker-assisted selection, based on the targeted use of molecular genetic markers, has been successfully applied to markedly reduce the impact of this disease.
However, despite several QTL studies in aquaculture species and ample evidence for the heritability of disease resistance traits, only a handful of large-effect QTL have been detected, and most disease resistance and other production-relevant traits are underpinned by a polygenic (multiple genes) genetic architecture. As such, genetic improvement of disease resistance relies on family-based selective breeding programs, augmented by the use of genomic selection, for which disease resistance has been a major focus.
The substantial opportunity for genetic improvement of disease resistance and other performance traits in aquaculture species, combined with initial success of in vivo genome-editing trials, opens exciting new avenues to improve aquaculture production and sustainability. There are three main categories by which genome-editing technology could be applied to make step changes in genetic improvement, and each requires different approaches to the underpinning research leading to discovery of functional alleles: (i) detecting, promoting, removing, or fixing targeted functional alleles at single or multiple QTL(s) segregating within current broodstock populations of a selective breeding program; (ii) targeted introgression-by-editing of favorable variants from different populations, strains, or species to introduce or improve novel traits in a population; and (iii) creating and utilizing de novo favorable alleles that are not known to exist elsewhere. We discuss each of these avenues, and a unique opportunity to harness a combination of in vivo and in vitro approaches to understand and improve disease resistance in aquaculture species is presented.
Source : Global Aquaculture Alliance